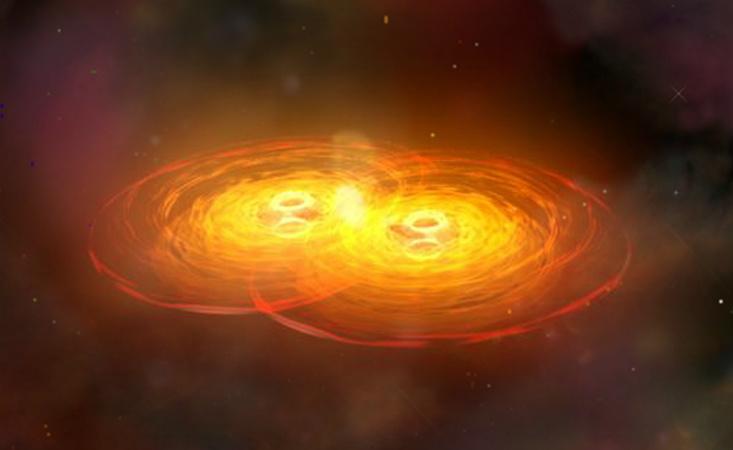
The galaxy known prosaically as M87 doesn’t look like much. Unlike beautiful spiral galaxies (including the Milky Way), M87 appears as an orangish blob of stars through telescopes. Its only noticeable feature is the long streamer of gas emanating from the galactic center.
The source of that jet is far from prosaic, however: It’s a black hole 6.6 billion times the mass of the Sun. No other known object is as massive—this black hole by itself outweighs entire star clusters and small galaxies. Even compared to other huge black holes, such as the one at the heart of the Milky Way, the monster of M87 is immense.
But how did these black holes grow so massive? The simple answer: Just as big galaxies grew by colliding and merging (as described in Steve Nadis’ recent Nautilus piece, “The Stories That Galaxies Tell”), the largest black holes form when pairs of smaller black holes merge. Trying to grasp the story in greater detail pushes both our theoretical and observational limits: Colliding black holes demand complex computer simulations to understand and sophisticated machines to detect. Studying black hole coalescence may be the best way to understand the effects of absurdly strong gravity, potentially revealing entirely new phenomena.
Black holes seem to have a close connection with their host galaxies, hinting at their shared evolutionary history. The size of a black hole, for example, seems to mirror the size of the central region of its galaxy. Astronomers do not expect to see “super-massive” black holes—those with masses millions or billions of times greater than the Sun—in tiny galaxies or vice-versa (though at least one seems to violate the rule for unknown reasons).
Since the largest galaxies formed out of mergers between smaller galaxies, researchers suspect the same applies to their central black holes. Galaxies showing the signs of recent collisions, such as NGC 6240, sometimes also have two obvious super-massive black holes, lending support to the idea. But the process of turning two black holes into one takes many millennia, far longer than humans have been engaged in astronomy. So the only way we currently have to understand close encounters of black holes is with theory.
Theoretical models show that during galactic mergers, black holes fall into mutual orbit, starting with wide separation and gradually spiraling in toward each other. General relativity predicts that two black holes will radiate energy away in the form of gravitational radiation as they draw ever closer, until eventually they coalesce.
The galaxy known prosaically as M87 doesn’t look like much. The source of a jet emanating from that galaxy is far from prosaic, however: It’s a black hole 6.6 billion times the mass of the Sun.
Just as rapidly changing electric currents produce electromagnetic radiation—in the form of radio waves—the motion of masses can produce gravitational radiation. However, gravitational radiation is far harder to detect than radio waves because gravity is much weaker than the electromagnetic force. Gravitational waves are presumably all around, but they pass through us unnoticed. The 1993 Nobel Prize in Physics was awarded to Russell Hulse and Joseph Taylor for their observation that binary pulsars behaved exactly as predicted if they were emitting gravitational radiation. But direct detection still eludes us.
Even Earth emits gravitational waves as it orbits the Sun, though the amount of energy lost is imperceptible over the lifetime of the Solar System. Binary black holes are a different matter: Once they are relatively close, they shed a tremendous amount of energy, bringing them closer together with each orbit. (Binary black stars are thought to emit more gravitational energy as they merge than regular stars emit in the form of UV, IR, and visible light over their entire lifetimes of billions of years.) Eventually their event horizons will touch, and the system emits a lot more gravitational waves in a phase known as “ring-down,” as the lumpy, uneven merged mass becomes a smooth, perfectly symmetrical black hole.
Unfortunately, that smooth black hole contains no information about its lumpy past: We can’t tell by looking at a black hole if it formed out of a merger or if (to quote the famous theoretical physicist Lady Gaga) it was born that way. However, all the lumpiness, the misalignments, the complexity of what happened during the collision are encoded in the gravitational-wave signal.
And that’s why the gravitational wave signal is so important: It may be the best means we have to study black hole mergers—provided we can catch coalescence in the act. That’s the goal of experiments such as the ground-based Laser Interferometer Gravitational-wave Observatory (LIGO), which was completed in 2010 and is scheduled to start again, with better detectors, in 2014; and the projected space-based Laser Interferometer Space Antenna (LISA), which has unfortunately been delayed and dramatically reduced in scope thanks to budgetary troubles. Ground- and space-based gravitational wave observatories have complementary jobs in this mission: The orbiting facilities would be able to detect radiation from black hole binaries far from coalescence, while earthbound experiments could catch the final moments before the merger, the collision itself, and the ring-down.
Theoreticians, meanwhile, are not just waiting to see results roll in, but refining their models of this process, characterizing the shape gravitational waves should take based on Einstein’s theory of relativity. That’s important for two reasons. First, it may be possible to see gravitational radiation from a black-hole collision that’s hidden from ordinary telescopes. Second, if the signal observed at LIGO or LISA deviates from predictions, theory could tell us if something new and interesting is going on.
Astronomers have no way to probe inside black holes, or even to see close to their event horizons. But merging black holes could reveal the operations of gravity at its most extreme—and tell us exactly how the biggest black holes in the Universe got their start.
Matthew Francis is a physicist, science writer, public speaker, educator, and frequent wearer of jaunty hats. He’s currently writing a book on cosmology with the working title Back Roads, Dark Skies: A Cosmological Journey.