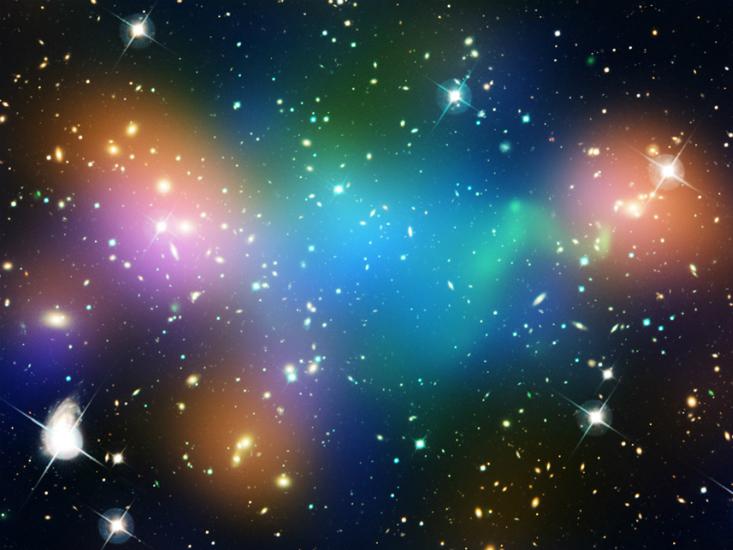
If I told you that I was 99.81 percent certain I had made a big discovery, you might suggest it was time to break out the champagne. If I said the discovery resolved one of the biggest outstanding problems in science and would probably let me punch a ticket to Stockholm to pick up a Nobel Prize, you might suggest ordering a case of the best champagne I could find, and start planning out my acceptance speech. After all, 99.81 percent certainty is pretty good; it’s well above 95 percent, the bar used for “statistical significance” in most fields of research.
But most physicists would tell me to hold off on the bubbly until I got rid of a bit more of that remaining .19 percent uncertainty. How much more? Well, the simple answer would be, “almost all of it.”
Dark matter is an enigmatic substance that accounts for most of the universe’s mass and has shaped its entire history—we quite literally wouldn’t be here without it. Yet years of searching for dark matter particles have left us with no idea what the stuff looks like, much to the intense frustration of many physicists. Discovering it, and knowing its properties, could help fill in many details about how the universe got to its present state.
So, you might think scientists would get pretty excited about the recent announcement about a potential dark matter sighting that scientists were 99.81 percent certain didn’t arise by chance. Unfortunately for the people who did the sighting, claiming discovery in physics, and with dark matter in particular, turns out to be a very complicated thing.
Tantalizing hints
Dark matter picked up its name not because it’s black, but because we can’t see it. The puzzle emerged when astronomers calculated that there wasn’t enough matter to produce the gravitational force necessary to keep galaxies rotating and draw them together into giant clusters the way they do. They hypothesized there must be some matter we do not see producing the extra tug of gravity. Since it’s not visible, we know that it doesn’t interact with light or other forms of radiation. And, in fact, it can’t interact with regular matter much either, or we’d have seen it slam into our detectors by now. But it does create gravity, and its gravitational pull has been influencing the evolution of the Universe since shortly after the Big Bang. Since things were too hot for atoms to exist back then, scientists decided dark matter must be a particle, and gave it a nickname: WIMP, for weakly interacting massive particle.
If WIMPs don’t interact with light or other particles, how could you possibly detect one? According to some models of dark matter’s behavior, the whole “doesn’t interact with regular matter” idea is only an approximation. Very, very rarely, a dark matter particle could have a very, very weak interaction with normal matter. Rather than slamming into a detector, a dark matter particle might sometimes give one of its atoms a gentle nudge. A number of teams have built specialized detectors that are sensitive to these very low-energy nudges.
One of those teams is the Super Cryogenic Dark Matter Search (SuperCDMS). They stuck a number of extremely sensitive detectors deep in a mine to get them away from all the energy that would bombard them on the Earth’s surface. The hardware was chilled to near absolute zero so that the vibrations of the atoms in the detectors themselves didn’t create false signals. The team learned to identify the stray electrons and neutrons that filtered in from the radioactive decay of atoms in the mine’s walls. Then they waited for several years.
In that time, they picked up just three events [pdf] that looked like the impacts of dark matter particles. But their calculations said they shouldn’t have seen any at all, and that there was only a .19 percent chance of the three events coming from random noise in their equipment. (The researchers reported a 5 percent chance of seeing three impacts, and a .19 percent random chance of seeing three impacts clumped closely together, as they did.) Pretty exciting, right?
Reaching for certainty
Unfortunately, physicists have been wrong about 99 percent certainties in the past—they’ve simply vanished when more data came in. These certainties can be expressed in terms of a statistical measure called standard deviation, abbreviated as σ, or sigma. By the conventions of particle physics, to announce a discovery, a finding has to be five sigma: There’s only a 0.0001 percent of seeing similar results if there was no real signal to detect, and the researchers were just looking at a collection of random noise.
SuperCDMS’ 99.8 percent certainty is only about three sigma.
And, crucially, that value assumes the team did everything right. That’s never guaranteed, as demonstrated by the recent case where a loose cable connection made it look like some particles called neutrinos were moving faster than the speed of light. Those sorts of hardware errors are joined by the possibility of going astray on theoretical grounds. To get their results, the authors had to make models of how every type of potential noise—random radioactive decays, stray electrons, and so on—would look like in their detector, and how often they’d see them.
Although the team behind SuperCDMS is filled with very smart people, this process involves a number of assumptions and approximations—there’s always the chance they didn’t think of one complicating factor or another. Until the signal gets stronger, those assumptions won’t even be regarded as significant enough for the rest of the physics community to look hard at the data and see if it’s accurate and important.
Crossed signals
SuperCDMS’ result is in good shape in one regard. Just across the mine shaft is a separate dark matter detector called CoGeNT, and it reported seeing a signal that reaches 2.8 sigma. Critically, the two detectors roughly agree on the mass of the particle. Expressed as its equivalent in energy (as Einstein pointed out, the energy and mass of a particle are directly connected), CoGeNT sees a dark matter particle with a mass of 7 gigaelectronvolts. The SuperCDMS particle appears to weigh in at about 8.6 GeV. That’s not identical, but it’s close enough that the two detectors could be seeing the same thing.
A similar mass has been suggested by a detector based in Italy called DAMA/LIBRA. Using a completely different technology, it sees a seasonal variation in particle detections that may reflect the Earth’s orbit pushing it against a flow of dark matter each spring.
That’s the good news. The bad news is that a different detector, called Xenon-100, seem to rule this out entirely. And that’s where things get confusing. The CoGeNT team doesn’t think that Xenon-100 really has fully ruled things out. The Xenon-100 team doesn’t believe the CoGeNT results. CDMS researchers have largely stayed out of the debates. And nobody seems to trust the DAMA/LIBRA results, in part because the team behind the detector has been cagey about the experimental details.
There are also some experiments in space that have relevant data, but they don’t make things any clearer. It’s thought that dark matter particles can sometimes collide with themselves, leaving energetic antimatter in the wreckage. A satellite-based detector called PAMELA initially seemed to produce evidence of this antimatter but, after gathering more data, the signal disappeared (and in any case, the particle was much heavier than 8GeV). The same thing happened to the Fermi space-based telescope, which saw evidence of a 130GeV particle fade into the background when more data came in. Meanwhile, a particle detector on the International Space Station has seen lots of antimatter, but not seen any dark matter signal in it.
If all of these seemingly contradictory results confuse you a bit, don’t worry—they probably confuse a lot of physicists as well. Ultimately, any dark matter finding will have to make some sense out of most of them. Each experiment has to deal with not only its own uncertainty, but the uncertainties of the field as a whole.
Evidence confronts theory
Because dark matter influences the evolution of the universe, the universe itself can also tell us whether our experiments are on the right track. A particle with a mass in the area of the one seen by CoGeNT and SuperCDMS (about 8GeV) is considered a “light” particle, since it’s about 10 times less than the mass of particles like the W, Z, and Higgs. And scientists also have a lot of reasons to dislike a light dark matter particle.
Some of these are simply practical; a light particle will produce a smaller signal in detectors, making it easier to confuse with background noise. But others are purely theoretical. For example, the leading candidate to explain any particles beyond the ones we know about is called supersymmetry. Most of the supersymmetric particles are unstable, but the lightest (a cousin of the neutrino called the “neutralino”) should be stable, and it would make an excellent dark matter particle. But the neutralino is expected to be relatively heavy. Of course, it’s also still theoretical; the Large Hadron Collider is rapidly narrowing down the space where we might hope to find a neutralino, with no hint of a signal yet.
The universe itself may also be telling us to think heavy. Even though dark matter only interacts via gravity, those interactions have been enough to create the structures of the Universe. For that to work, the dark matter has to be moving slowly enough for gravity to grab hold of it—in physics terms, it has to be “cold.” But the WIMPs stopped interacting with anything very shortly after the Big Bang, when the Universe was very hot, and they’ve had no way to lose that heat since. This apparent contradiction is easiest to resolve if the particle is massive. Even if it carries lots of energy, the particle’s mass means it will still be moving slowly enough for gravity to grab hold of it.
How do you actually resolve this sort of confusion? The traditional route to particle discovery, through accelerators like the Large Hadron Collider, provides a good example. All of the big accelerators used for particle detection incorporate two detectors, built using different technologies, so that any flukes related to the hardware get controlled for. Until physicists see a significant signal using both of them, they tend to consider matters as not being settled. (The Higgs boson, for instance, was discovered by two different detectors at the LHC.) That attitude is likely to carry over to dark matter, with many waiting for signs of a discovery on two independent detectors before getting confident we’ve solved it.If that does happen, the rest of physics will adjust. The teams that thought they’d ruled out the existence of the particle will go back and try to figure out what they did wrong. The astronomical results will be reanalyzed to figure out why there were no obvious signals. And theoreticians will figure out which of their many models can be adapted to make sense of the new results, giving up on supersymmetry if it turns out to be a bust. Cosmologists would find a way to make a lighter WIMP work if they needed to.
But, until there’s better evidence—a signal much stronger than three sigma, confirmation from at least one other experiment, and some explanations for the contradictory evidence from other experiments—it seems too early to conclude that that mystery of dark matter has been solved.
John Timmer is the Science Editor at Ars Technica. He also teaches scientists how to communicate with the public and each other at Cornell Medical College, Stony Brook University, and other institutions.